Abstract
The immune system possesses the capability to identify tumor cells and eradicate early malignant tumor cells. Thus, activating the immune system of cancer patients provides great therapeutic benefits. Inhibitory T-cell immune checkpoints play a vital role in tumor immune escape. Thus, immune checkpoint inhibitors (ICIs) have attracted attention in cancer immunotherapy. In ICI therapy, the therapeutic targets are the expressed immune checkpoints of T cells. Immune checkpoints induce T-cell dysfunction in cancer. However, ICIs or immunomodulators restore the antitumor actions of cytotoxic T cells by blocking immune checkpoints. ICIs have become desirable treatment options because of their broad range of activities and response rates ranging from 15% to 90% in several cancer types. Generally, ICIs also have favorable toxicity profiles. This paper will first delve deeper into the best-known immune checkpoints and then review ICIs that are attractive treatment options in immunotherapy.
Introduction
Cancer is considered the second leading cause of death of human beings worldwide after cardiovascular disease, and despite tremendous scientific developments, the cure for cancer is still challenging1. The reasons behind this include lifestyle, method of eating, societal change, industrialization, environmental pollution, and inadequate treatment options1, 2. Among the different etiological factors contributing to the genesis of cancer, societal modernization is regarded as a leading factor1, 2, 3, 4. It is estimated that the number of deaths from cancer may increase to 11 million by 20301, 5.
Treatment options such as surgery are effective for localized cancers only. Radiotherapy can play a vital role in cancer treatment if the cancer is not disseminated5. Chemotherapy is considered one of the primary treatment options for cancer. To date, various chemotherapeutic agents have been developed and are being used either alone or in combination to treat cancer5, 6. Also, the discovery of the chemotherapeutic agent, cisplatin, and the development of its various analogs have created optimism in chemotherapy1, 4. However, the side effects of these chemotherapeutic agents restrict their successful use1, 3, 5. Again, nanoparticles, such as ZnO, have gained increased attention as chemotherapeutic agents because of their targeted action and minimal side effects5. Despite significant advances in understanding cancer etiology, ideal anticancer drugs or strategies are still missing1, 3.
Established tumors require the employment of diverse mechanisms to suppress the antitumor response. The mechanisms include the upregulation of coinhibitory molecules called immune checkpoints, engaging immunosuppressive immune cells and inhibitory cytokine production7. Nevertheless, the immune system possesses the capability to identify tumor cells and eradicate early malignant tumor cells8. Thus, activating the immune system of cancer patients for therapeutic benefit has long been pursued by scientists9. Research on inhibitory T-cell immune checkpoints has realized this goal10. Furthermore, recent studies have shown that inhibitory T-cell immune checkpoints play a vital role in tumor immune escape. Thus, immune checkpoint inhibitors (ICIs) have attracted attention in cancer immunotherapy11. As a result, the past decade has observed a quick transition in cancer treatment with the advent of immunotherapy12.
Immunotherapy is one oncologic treatment type undertaken to enhance host immunity to fight cancer13. It harnesses the immune system’s capability to identify nonself-tumor antigens and constantly adapt to and detect new antigens14. In addition, it targets the development of tumor rejection capabilities, breaking the tumor-induced immune tolerance15. The advantages of immunotherapy include sustained surveillance, a low toxicity profile, and the ability to detect a small number of cancer cells16. Thus, immunotherapy is regarded as an alternative method of cancer treatment. Furthermore, the success of ICIs suggests that active immunotherapy can contribute to obtaining a longer-lasting response in cancer patients10, 11, 17. In this paper, ICIs and their role in immunotherapy are discussed in depth with their prospects in medical science.
Literature research was performed in PubMed and Google Scholar with the following search terms: “immune checkpoint inhibitor” and “cancer” in combination with “melanoma,” “metastasis,” “ICI,” “PD-1,” “CTLA-4,” “LAG-3,” “TIM-3,” “BTLA,” “PD-L1,” “PD-L2,” and “toxicology.”
Immune Checkpoints
Tumor-infiltrating lymphocytes (TILs), a kind of naturally occurring T cells, can identify tumor antigens13, 18. However, TILs, especially CD8+ T cells, cannot successfully eliminate cancerous cells when the effector function of infiltrating T cells is curtailed by immunosuppressive mechanisms of the tumor microenvironment19. Among these mechanisms, the upregulation of immune checkpoints has emerged as a major marker for the dysfunction of T cells in cancer20. Thus, in ICI therapy, the therapeutic targets are the expressed immune checkpoints of the T cells. Immune checkpoints are coinhibitory or costimulatory molecules that induce T-cell dysfunction in cancer. However, ICIs or immunomodulators restore the antitumor actions of cytotoxic T cells by blocking immune checkpoints21. ICIs have become very appealing treatment options because of their broad range of activities and response rates ranging from 15% to 90% in several cancer types. Generally, ICIs also have favorable toxicity profiles22.
The best-known immune checkpoints corresponding to T-cell dysfunction along with controlling immune responses include PD-1 (Programmed Death 1), CTLA-4 (Cytotoxic T-lymphocyte-associated Antigen 4), LAG-3 (Lymphocyte-activation Gene 3), TIM-3 (T-cell Immunoglobulin and Mucin Domain 3), and BTLA (B- and T-lymphocyte Attenuator)20, 21, 23. These best-known immune checkpoints will be discussed in depth in the following sections.
PD-1
PD-1 is a significant controller of tumor T-cell effector functions24. This CD28 homolog is expressed in activated T cells, natural killer (NK) T cells, dendritic cells (DCs), and activated B cells, to name a few. Furthermore, expression is induced by interleukin (IL)-21, IL-15, IL-7, and IL-2 on T cells16, 21, 25, 26, 27, 28. It was isolated from a T-cell hybridoma that was experiencing T-cell receptor (TCR) activation-induced cell death. Thus, it was called PD-19, 29. This type I transmembrane protein comprises an intracellular domain with an ITIM (immunoreceptor tyrosine-based inhibitory motif) along with an ITSM (immunoreceptor tyrosine-based switch motif), a transmembrane domain, an immunoglobulin (Ig) superfamily domain, and a stalk of ~20 amino acids (aa)11, 30. The tyrosine of ITSM is necessary for PD-1 functioning in B and T cells28. When ITIM and ITSM become phosphorylated, they recruit Src homology 2 (SH2)-containing tyrosine phosphatase 1 (SHP-1) along with SH2-containing tyrosine phosphatase 2 (SHP-2). SHP-1 and SHP-2 reduce TCR signaling by dephosphorylating the CD3zeta chain21, 30.
On mouse chromosome 1 and human chromosome 2, PD-1 is produced by the Pdcd1 gene. In both humans and mice, Pdcd1 is composed of 5 exons. A short signal sequence and an Ig domain are produced by exons 1 and 2, respectively. Exon 3 is composed of the transmembrane domain and the stalk. A short, 12 amino acid sequences are encoded by exon 4, indicating the cytoplasmic domain’s outset. Exon 5 contains long 3’ UTR and C terminal intracellular residues30. The biological significance of PD-1 pervades the immune response, such as tumor immunity, autoimmunity, transplantation immunity, infectious immunity, and allergy31.
PD-L1 is, in fact, a PD-1 ligand11, 30. This type 1 transmembrane protein is also called CD274 and B7-H18. In humans, it is expressed by, among others, macrophages, vascular endothelial cells, astrocytes, pancreatic islet cells, T cells, DCs, and B cells32. On mouse chromosome 19, PD-L1 is produced by the Cd274 gene. However, in human chromosome 9, it is produced by the Pdcd1 gene. CD274 consists of 7 exons. Exon 1 possesses a 5’ UTR, and exon 2 possess a signal sequence. Again, exons 3 and 4 possess an IgV-like and an IgC-like domain, respectively. Furthermore, exons 5 and 6 possess a transmembrane and intracellular domain, respectively. Exon 7 contains a 3’ UTR and ~30 aa long intracellular residues30.
PD-L2 is also a PD-1 ligand30. This type 1 transmembrane protein is also termed B7-DC or CD2738, 30. Its expression is limited to DCs, macrophages, and B cells and is encoded by the gene Pdcd1lg2 neighboring the gene Cd274 32. In mice, the Pdcd1lg2 gene consists of 6 exons, while it consists of 7 in humans. Exon 1 is noncoding, and exon 2 possesses a signal sequence. Furthermore, the IgV-like and IgC-like domains are produced by exons 3 and 4, respectively. Exon 5 consists of a transmembrane region, a short stalk, and the outset of the cytoplasmic domain. Exon 5 in mice possesses a stop codon that produces a 4 aa long cytoplasmic domain. Finally, the additional coding region in humans (exon 6 and 7) results in a 30 aa long cytoplasmic domain30.
CTLA-4
CTLA-4, or CD152, is a significant controller of T-cell activation21, 24. This homolog of TCR CD28 is expressed on activated B cells in addition to activated CD4+ and CD8+ T-cell surfaces21, 33. It binds to ligands CD80 (also termed B7-1) and CD86 (or B7-2) with increased affinity compared to CD288, 33, 34, 35. CTLA-4 binding with ligands with higher affinity than CD28 decreases CD28-dependent costimulation. Again, CTLA-4 can mediate inhibitory effects on the major histocompatibility complex (MHC)-TCR pathway directly21, 36. CTLA-4 can recruit SHP-2 along with PP2A to the intracellular YVKM domain. SHP-2 attenuates TCR signaling by dephosphorylating the CD3zeta chain. PP2A impairs TCR signaling by inhibiting downstream Akt phosphorylation21, 24, 32. Actually, CTLA-4 was isolated from a mouse T-cell cDNA library. Hence, it was named CTLA-4, comprised of 3 introns and 4 exons, and encodes a type I transmembrane protein34. CTLA-4 also shares a conserved motif of the MYPPPY amino acid sequence with CD28. This conserved motif is thought to be critical for ligand binding34, 36, 37.
LAG-3
LAG-3, another type I transmembrane protein, is associated with the Ig superfamily and possesses 4 Ig-like domains38. This CD4-related inhibitory receptor is also known as CD223. It has a higher binding affinity for MHC II than for CD4, and is expressed in NK cells, activated CD4+ and CD8+ T cells, B cells, TILs, and T-regulatory cells (Tregs). On tolerant TILs, LAG-3 is coexpressed with PD-1. It further suppresses the activation of antigen-presenting cell (APC) binding with MHC II molecules8, 16, 24, 39. Again, it inhibits CD4+ activation and decreases CD8+ cytotoxic function20. This inhibitory function is dependent on signaling through the cytoplasmic domain motif KIEELE. In addition, LAG-3 transfection into T cells can provide a regulatory function. Thus, it is essential for the maximal function of Tregs8. However, the exact molecular mechanism of downstream signaling of LAG-3 is not entirely known38.
TIM-3
TIM-3, another type I transmembrane protein, is expressed on monocytes, DCs, T cells, Tregs, and macrophages20, 39, 40. Furthermore, it is found in activated human NK cells and suppresses the cytotoxicity of NK cells41. TIM-3 is associated with carcinogenesis, as its expression correlates with reduced survival, tumor invasion, and metastasis. It can be coexpressed with PD-1 on APCs20. Several ligands, such as phosphatidylserine, CEACAM-1, galectin-9, and HMGB1, have been reported for TIM-38. Also, TIM-3 causes CD4+ and CD8+ T cell apoptosis upon galectin-9 binding through the calcium-calpain-caspase-1 pathway20. Together with PD-1 and other inhibitory receptors, it can mediate the exhaustion of CD8+ T cells41.
BTLA
BTLA, or CD272, a glycoprotein of the Ig superfamily, has been identified as an inhibitory receptor20, 42, 43, 44. This type I transmembrane cosignaling receptor is structurally connected with CTLA-4 and PD-140, 45. It is expressed on macrophages, DCs, T cells, B cells, and NK cells20, 46. In its cytoplasmic domain, it contains ITIMs and recruits both SHP-1 and SHP-2 upon phosphorylation. In addition, it negatively regulates both TCR and B-cell receptor signaling in vitro 42, 44, 46. For example, the herpes virus entry mediator (HVEM) transmits signals to BTLA-expressing cells and functions as a ligand 42. HVEM has 4 cysteine-rich domains in its extracellular region that mediate the binding of HVEM with BTLA. Upon binding, HVEM transmits signals by TNF receptor-associated factor 2 to induce STAT3 phosphorylation, which results in the activation of NF-kB and other prosurvival signals46. Again, the binding of BTLA with ligands decreases cytokine production and T-cell proliferation40. Thus, BTLA is considered a possible target in immunotherapy43. The interaction of various immune checkpoints with their respective ligands is summarized in Figure 1.
T-cell Activation
Two effector cells of adaptive immunity include cytotoxic T cells and helper T cells. Helper T cells contribute to the propagation of the antitumor immune response, and cytotoxic T cells kill tumor cells directly. T-cell activation is necessary for the functioning of both cell types7. T-cell activation is essentially a strongly controlled process47. For efficient T-cell activation, activation signals must be presented. To explain T-cell activation, a three-signal hypothesis has been formulated. Signal 1 is given by the interaction between the APCs of MHC and TCR20, 48. CD4 and CD8 coreceptors are related to TCR. CD4 binds with MHC II, whereas CD8 binds with MHC I49. Next, T-cell surface molecules provide a costimulatory signal that serves as signal 2. CD28 is the most effective and best-characterized costimulatory molecule associated with T-cell activation. This 44-kDa glycoprotein molecule binds to CD80 and CD86 on the APCs. Finally, cytokines act as signal 3 for the activation of T cells. However, CD28 signaling increases cytokine production by T cells and increases T-cell survival by upregulating BCL-XL, a member of the BCL-2 family of proteins20, 35, 48, 50.
Interdependency of ICI and T-cell Activation
PD-1 interaction with its ligands alleviates T-cell functions by restraining the effector activity of T cells against cancers9, 51, 52. Ligand binding with PD-1 causes tyrosine phosphorylation of the PD-1 cytoplasmic domain and recruits both SHP-2 and SHP-1. These events reduce the phosphorylation of TCR signaling molecules and decrease cytokine production and T-cell activation. PD-1 signals can decrease the expression of anti-apoptotic genes but increase the expression of proapoptotic genes. Additionally, PD-1 signals can also decrease the killing capacity of T cells by reducing their production of cytotoxic molecules8, 32, 40, 52, 53. Ligand binding to PD-1 also inhibits the PI3K/AKT pathway and downregulates Bcl-xl gene expression to enhance T-cell apoptosis11.
The PD-L2 ligand has a 3-fold increased affinity for PD-1 compared with PD-L1. However, its expression is restricted mainly to myeloid cells. Alternatively, PD-L1 is primarily expressed in nonhematopoietic as well as hematopoietic cells28, 54, 55. PD-1 engagement by PD-L2 prevents TCR-mediated proliferation along with cytokine production. Moreover, PD-L2 and PD-1 interaction inhibits B7-CD28 signals at lower antigen concentrations. However, at higher concentrations, these interactions reduce the production of cytokines but do not inhibit T-cell proliferation. These interactions are also found to arrest the cell cycle at the G0/G1 phase but do not increase cell death25.
CTLA-4 is a negative controller of the T-cell response, and it prevents the T-cell response in 2 ways8, 33, 34, 35, 56. First, it intrinsically inhibits T-cell activation by outcompeting CD28 to binding with the ligand B7 or reducing TCR and CD28 signaling, recruiting phosphatases to the CTLA-4 cytoplasmic domain. Second, CTLA-4 of one T-cell prevents the activation of another T-cell, lessening the expression of CD80 and CD86 on APCs8, 53, 54. Again, CTLA-4 can also inhibit TCR signal transduction by binding to the TCR zeta chain and preventing tyrosine phosphorylation56.
Blocking of Immune Checkpoints
Immune surveillance employs both the innate and adaptive immune systems and clears early malignant cells in cancer. However, tumors employ various mechanisms to escape this action of immune surveillance. For example, checkpoint blockade by ICIs has dramatically changed cancer treatment by activating the immune system of patients26. Checkpoint blockade in cancer immunotherapy uses antibodies to block the pathways that prevent responses of T cells to tumors8.
PD-1 and PD-L1 interaction blockade has exhibited a tremendous antitumor response57, 58. PD-1 pathway blockade has exhibited 30%-50% response rates in clinical trials for various cancers59. Even more, pembrolizumab, a member of the anti-PD-1 family, has gained the approval of the US Food and Drug Administration (FDA) in melanoma cancer treatment8, 9. Furthermore, in 2013, this humanized IgG4- κ monoclonal antibody (mAb), previously known as lambrolizumab and MK-3475, received the “Breakthrough Therapy” designation for advanced melanoma from the FDA21, 39, 60, 61. Pembrolizumab has also been successful in non-small cell lung cancer (NSCLC) treatment55. It blocks PD-1 and thus releases it from interactions with ligands and provokes an antitumor response40, 62.
Nivolumab (MDX-1106 or BMS-936558), an anti-PD-1 mAb, has also received approval from the FDA for cancers, such as squamous cell lung cancer, renal cell carcinoma, and melanoma treatment8, 9, 40, 53, 63. In 2014, this human IgG4- κ mAb achieved the “Breakthrough Therapy” designation from the FDA for non-Hodgkin’s lymphoma treatment21, 61. Nivolumab blocks the binding of PD-1 with its ligands and thereby augments the host antitumor response by attenuating inhibitory signals14, 64. As a result, nivolumab provides significant clinical activity and an acceptable safety profile in squamous NSCLC14. Additionally, both pembrolizumab and nivolumab prolong overall survival compared to the chemotherapy drug, docetaxel, and have received FDA approval as second-line treatments in advanced NSCLC65.
Pidilizumab, an IgG1- κ mAb blocks PD-1, and BMS-936559, an IgG4 mAb, prevents PD-L1 binding with CD80 and PD-121, 39. Again, MPDL-3280A is another IgG1- κ mAb to PD-L1. In its Fc region, it possesses a single amino acid substitution16, 39, 66. This monoclonal antibody has received approval from the FDA for NSCLC and bladder cancer treatment9.
The first clinically targeted immune checkpoint receptor is CTLA-424. CTLA-4 blockade involves the induction of long-term regression of tumors59. Anti-CTLA-4 antibodies increase the ratio of CD4+ and CD8+ effector T cells (Teffs) to forkhead box protein 3 (FoxP3)+ Tregs in tumor infiltrates, which is associated with the enhanced eradication of tumors8, 55, 67. An increased ratio of Teffs to Tregs is a hallmark of successful tumor rejection with CTLA-4 blockade68. Recent studies suggest that the therapeutic outcome of anti-CTLA-4 antibodies is possibly not just because of the CTLA-4 interaction blockade with ligands but also because of the lessening of intratumoral Tregs through Fc receptor-mediated cytotoxicity8, 66.
The first FDA-approved ICI was ipilimumab (MDX-010). This human IgG1- κ mAb blocks CTLA-4. The half-life of ipilimumab is 15.4 days66. Ipilimumab received FDA approval in 2011 for metastatic melanoma treatment9, 39, 61. It has been assessed in clinical trials with renal cell carcinoma, prostate cancer, and melanoma malignancies21. Additionally, this was the first ICI to be evaluated in NSCLC66. In addition, it has improved overall survival in advanced cutaneous melanoma15. It blocks the CTLA-4 receptor from interacting with the ligands B7-1 and B7-27, 55, 64. It also inhibits the signaling activity of CTLA-461. Tremelimumab, a humanized IgG2 mAb blocking CTLA-4, has also entered clinical trials, showing a durable response in early clinical trials21, 39, 40, 53, 66.
Various anti-LAG-3 antibodies are now being evaluated in clinical trials, with relatlimab being the first anti-LAG-3 antibody developed commercially38. Blocking LAG-3 inhibits Tregs and restores cytotoxic T-cell function20. Zhou et al. identified LBL-007 and reported that this novel humanized anti-LAG-3 antibody demonstrated a higher affinity for LAG-3 antigen and blocked downstream signaling and functions of LAG-3. They also reported that LBL-007 suppressed colorectal cancer cell growth in mice when used alone or with a PD-1 antibody38.
Unfortunately, the blocking mechanism of TIM-3 mAbs is not yet fully understood8. However, it has been found that targeting the Gal9/TIM-3 axis together with induction chemotherapy could effectively increase the possibility of total remission of acute myeloid leukemia. Again, combination therapy of PD-1 and TIM-3 mAb can regulate tumor growth in a synergistic way69. In melanoma and mammary carcinoma models, anti-BTLA antibodies have demonstrated significant antitumor activity44. Further, it has been found that the duel blockade of BTLA and PD-1 enhances antitumor immunity24 and releases immune exhaustion in tumor-specific T cells in melanoma patients. Hence, BTLA inhibition with other blocking antibodies is a possible therapeutic approach in cancer immunotherapy45. A list of the best-known ICIs for immunotherapy is presented in Table 1.
Immune Checkpoint Inhibitor (ICI) | Target Immune Checkpoint | Antibody Isotype | Appoval Status | References |
---|---|---|---|---|
Pembrolizumab | PD-1 | Ig G4 | FDA-approved | 8 , 9 , 21 , 39 , 60 , 65 |
Nivolumab | PD-1 | Ig G4 | FDA-approved | 8 , 9 , 21 , 40 , 53 , 61 , 63 |
MPDL-3280A | PD-L1 | Ig G1 | FDA-approved | 9 , 16 , 39 , 66 |
Ipilimumab | CTLA-4 | Ig G1 | FDA-approved | 9 , 39 , 61 |
Tremelimumab | CTLA-4 | Ig G2 | Under Assessment | 21 , 39 , 40 , 53 , 66 |
Combination Therapy
Combination therapy helps enhance various steps in the cancer-immunity cycle to establish an active microenvironment where ICIs can exert successful antitumor killing8. As ICIs are becoming a mainstream treatment option in cancer, different combinations of various ICIs or ICIs with other proven treatment options are being tested to convert nonresponders to responders. PD-1 blockade-associated toxicity is less than CTLA-4 and IL-2 blockade. However, PD-1 and CTLA-4 combination checkpoint blockades improve the response rate compared with single checkpoint blockades8, 9. Furthermore, a CTLA-4 and PD-1 combination checkpoint blockade allows tumor-specific T cells to accomplish effector functions and increases the infiltration of Teff. So, it increases the advantageous ratio of Teff-to-Tregs and causes the tumor-suppressive microenvironment to shift to the inflammatory microenvironment51, 61. Again, LAG-3 and PD-1 combination blockade also exhibited increased antitumor activity compared with their single checkpoint blockade8, 38. Nivolumab and ipilimumab combination therapy has proven to be the most active immunotherapy regarding response rate, along with median progression-free survival in melanoma treatment40, 55, 70. The combination of nivolumab and relatlimab is being assessed for immunotherapy in solid cancers38.
Cancer therapies, such as radiotherapy, oncogene-targeted therapy, or chemotherapy, can change the immunosuppressive tumor microenvironment and synergize with ICIs. Thus, various combinations of ICIs with other cancer therapies are being tested53. For example, clinical trials have assessed ipilimumab and radiotherapy54. Golden EB et al. reported the first abscopal response (radiotherapy-induced regression of tumors in lesions distant from a targeted site) with the combination of radiotherapy and ipilimumab in lung cancer patients. They reported that irradiating single liver metastasis in a lung cancer patient with ipilimumab led to a durable and complete response54, 68. Therefore, radiation therapy can emerge as an optimal partner for ICIs because of its ability to induce a response in nonresponsive patients.
For many years, chemotherapeutic agents have been used to control cancers that do not respond to either radiation or surgery6. In addition, chemotherapeutic agents can initiate T-cell activation and sensitize tumor cells for T-cell-mediated killing by inducing tumor-specific antigen release. These observations inspired combining chemotherapeutic agents with immunotherapy to enhance patient response rate39. As ipilimumab is an FDA-approved ICI, the possible role of ipilimumab in combination with chemotherapy, other targeted therapies, and immunotherapy is being evaluated40. Thus, ipilimumab has been evaluated as a combination therapy with chemotherapeutic agents in small cell lung cancer (SCLC) and NSCLC in clinical trials66, 68. When used alone, ipilimumab has virtually no effect on lung cancer. However, combined with chemotherapeutic agents, it seems to provide a modest benefit in NSCLC and SCLC71.
Small RNAs without coding potential are known as microRNAs or miRNAs. A network of microRNAs regulates the expression of immune checkpoints, directly or indirectly. MicroRNAs can target multiple immune checkpoints and mimic the therapeutic benefit of combined checkpoint blockade. Dragomir et al. hypothesized that microRNA combined with a checkpoint blockade could increase the efficacy of established monotherapies72.
Toxicity
The hallmark toxicity associated with ICIs includes immune-related adverse events (irAEs) from aberrant activation of autoreactive T cells against host tissue. Substantial morbidity or even mortality may be infrequently caused by irAEs22, 73. However, these toxicities differ significantly from conventional chemotherapeutic toxicities74. Multiple organs, such as the peripheral and central nervous system, kidney, liver, pancreas, eyes, and skin, can be affected by irAEs32, 75. Moreover, immune toxicities, such as dermatitis, pneumonitis, diarrhea, colitis, thyroiditis, and hepatitis, are very common with ICIs. Pneumonitis is very rare but a potential life-threatening irAE of CTLA-4 and PD-1 inhibitors26, 76, 77. In monotherapy, thyroid disorders are almost the same for CTLA-4 and PD-1 inhibitors but remarkably increase in combination therapy. Symptoms of thyroid disorders include fatigue, alopecia, constipation, and palpitations78.
In general, PD-1 inhibitors demonstrate fewer irAEs than CTLA-4 inhibitors76, 79. However, thyroid dysfunction is higher in cases of anti-PD-1 antibodies (e.g., pembrolizumab and nivolumab)80, 81. Commonly reported adverse events of PD-1 inhibitors include rash, pruritus, fatigue, constipation, diarrhea, and arthralgia. Dry mouth and oral mucositis are more frequent with PD-1 inhibitors74. Pneumonitis is a very concerning toxicity associated with nivolumab66. On the other hand, hypophysitis has been reported as a characteristic side effect associated with CTLA-4 inhibitors. If not promptly identified, this situation can be life-threatening owing to secondary adrenal insufficiency15, 78. Common reported irAEs of ipilimumab include hepatotoxicity, diarrhea, endocrinopathies, and dermatologic toxicity74. Again, hypophysitis is frequently reported with ipilimumab80. Thyroid dysfunction is very common for pembrolizumab, but hypophysitis is found to be infrequent. Again, thyroid disorders are frequently reported irAEs for tremelimumab82. It has been found that irAEs with CTLA-4 blockade increase as the dose increases. However, irAEs with PD-1 blockade are not related to the dose7, 79, 82.
Toxicity associated with combination therapy is a matter of great concern76. Colitis is severe and more frequent with combination therapy83. Combination therapy of ipilimumab and nivolumab has demonstrated higher irAEs than their use in monotherapy. For combination therapy with ipilimumab with nivolumab, rash is the most commonly reported toxic effect, and this combination shows more frequent thyroid dysfunction and sometimes a higher grade76, 80.
Biomarker
PD-1 or PD-L1 monotherapy is considered well tolerated, but irAEs increase in combination therapy. Therefore, the development of biomarkers can contribute to maximizing therapeutic benefit, minimizing irAEs, and guiding combination therapy84. Predictive biomarkers help to determine the result of therapy before starting it. These can indicate whether a patient would benefit from monotherapy or if there is a need for combination therapy85. PD-1 is expressed on the activated T-cell surface. Therefore, it can be used as an activation marker86. Again, PD-L1 expression on specimens of pretreatment tumors can be a predictive biomarker with inhibitors of PD-139, 84, 87. Detection of PD-L1 with immunohistochemistry is thus far a commonly detected clinical biomarker to predict anti-PD-1/PD-L1 therapy response86.
Again, the status of baseline TILs and the mutational or neoantigen burden have been evaluated as predictive biomarkers in immunotherapy. In addition, peripheral blood marker testing is a noninvasive source of possible biomarkers in ICI therapy. However, no predictive peripheral blood marker has yet been validated84.
Conclusion
ICIs offer a blossoming field in cancer treatment. It has already shown valuable clinical benefits in several tumor types. Again, combination blockade by checkpoint inhibitors can exert better patient response rates. Therefore, a combination blockade of different checkpoint inhibitors needs to be tested. Although ICIs show less toxicity than previous immunotherapies, further study is required for a complete understanding of the side effects of these treatments. Further challenges in ICI therapy include exploring new therapeutic targets, optimizing dosing regimens, and identifying and validating biomarkers for predicting toxicity and clinical responses. Identifying reliable biomarkers will help make better treatment decisions regarding efficacy and toxicity. We hope the current manuscript will help us understand the basic principle of ICI therapy and its prospects in cancer treatment.
Abbreviations
aa: Amino Acid, APC: Antigen-presenting Cell, BTLA: B- and T-lymphocyte Attenuator, CTLA-4: Cytotoxic T-lymphocyte-associated Antigen 4, DC: Dendritic Cell, FDA: US Food and Drug Administration, FoxP3: Forkhead Box Pprotein 3, HVEM: Herpes Virus Entry Mediator, ICI: Immune Checkpoint Inhibitor, Ig: Immunoglobulin, IL: Interleukin, irAEs: Immune-related Adverse Events, ITIM: Immunoreceptor Tyrosine-based Inhibitory Motif, ITSM: Immunoreceptor Tyrosine-based Switch Motif, LAG-3: Lymphocyte-activation Gene 3, mAb: Monoclonal Antibody, MHC: Major Histocompatibility Complex, NK: Natural Killer, NSCLC: Non-small Cell Lung Cancer, PD-1: Programmed Death 1, SCLC: Small Cell Lung Cancer, SH2: Src Homology 2, SHP-1: SH2-containing Tyrosine Phosphatase 1, SHP-2: SH2-containing Tyrosine Phosphatase 2, TCR: T-cell Receptor, Teffs: Effector T Cells, TIL: Tumor-infiltrating Lymphocyte, TIM-3: T-cell Immunoglobulin and Mucin Domain 3, Tregs: T-regulatory Cells
Acknowledgments
None.
Author’s contributions
The manuscript was prepared by AS and MBM, AnS created the figure and edited the final manuscript. CM conceptualized the idea of this manuscript and supervised the review work. All the authors have read and approved the final manuscript.
Funding
None.
Availability of data and materials
Not applicable.
Ethics approval and consent to participate
Not applicable.
Consent for publication
Not applicable.
Competing interests
The authors declare that they have no competing interests.
References
-
Ali
I.,
Wani
W.A.,
Haque
A.,
Saleem
K.,
Glutamic acid and its derivatives: candidates for rational design of anticancer drugs. Future Med Chem.
2013;
5
(6)
:
961-78
.
View Article PubMed Google Scholar -
Ali
I.,
K. Saleem,
H.Y. Aboul-Enein,
A. Rather,
Social aspects of cancer genesis. Can. Ther.
2011;
8
:
6-14
.
-
Ali
I.,
Wani
W.A.,
Saleem
K.,
Hsieh
M.F.,
Anticancer metallodrugs of glutamic acid sulphonamides: in silico, DNA binding, hemolysis and anticancer studies. RSC Adv.
2014;
4
(56)
:
29629-41
.
View Article Google Scholar -
Saleem
K.,
Wani
W.A.,
Haque
A.,
Lone
M.N.,
Hsieh
M.F.,
Jairajpuri
M.A.,
I. Ali,
Synthesis, DNA binding, hemolysis assays and anticancer studies of copper(II), nickel(II) and iron(III) complexes of a pyrazoline-based ligand. Future Med Chem.
2013;
5
(2)
:
135-46
.
View Article PubMed Google Scholar -
A. Hussain,
M. Oves,
M.F. Alajmi,
I. Hussain,
S. Amir,
J. Ahmed,
M.T. Rehman,
H.R. El-Seedi,
Ali
I.,
Biogenesis of ZnO nanoparticles using Pandanus odorifer leaf extract: anticancer and antimicrobial activities. RSC Adv.
2019;
9
(27)
:
15357-69
.
View Article PubMed Google Scholar -
Sarder
A.,
Rabbani
M.G.,
Chowdhury ASMHK and Sobhani M. Molecular basis of drug interactions of methotrexate, cyclophosphamide and 5-fluorouracil as chemotherapeutic agents in cancer. Biomed Res Ther.
2015;
2
:
196-206
.
View Article Google Scholar -
La-Beck
N.M.,
Jean
G.W.,
Huynh
C.,
Alzghari
S.K.,
Lowe
D.B.,
Immune checkpoint inhibitors: new insights and current place in cancer therapy. Pharmacotherapy.
2015;
35
:
963-76
.
View Article PubMed Google Scholar -
Baumeister
S.H.,
Freeman
G.J.,
Dranoff
G.,
Sharpe
A.H.,
Coinhibitory pathways in immunotherapy for cancer. Annu Rev Immunol.
2016;
34
:
539-73
.
View Article Google Scholar -
Mahoney
K.M.,
Freeman
G.J.,
McDermott
D.F.,
The next immune-checkpoint inhibitors: PD-1/PD-L1 blockade in melanoma. Clin Ther.
2015;
37
:
764-82
.
View Article Google Scholar -
Mellman
I.,
Coukos
G.,
Dranoff
G.,
Cancer immunotherapy comes of age. Nature.
2011;
480
:
480-9
.
View Article Google Scholar -
Qi
X.,
Jia
B.,
Zhao
X.,
Yu
D.,
Advances in T-cell checkpoint immunotherapy for head and neck squamous cell carcinoma. Onco Targets Ther.
2017;
10
:
5745-54
.
View Article Google Scholar -
Pauken
K.E.,
Wherry
E.J.,
Overcoming T cell exhaustion in infection and cancer. Trends Immunol.
2015;
36
:
265-76
.
View Article Google Scholar -
Marin-Acevedo
J.A.,
Soyano
A.E.,
Dholaria
B.,
Knutson
K.L.,
Lou
Y.,
Cancer immunotherapy beyond immune checkpoint inhibitors. J Hematol Oncol.
2018;
11
(1)
:
8
.
View Article PubMed Google Scholar -
Rizvi
N.A.,
Mazières
J.,
Planchard
D.,
Stinchcombe
T.E.,
Dy
G.K.,
Antonia
S.J.,
Activity and safety of nivolumab, an anti-PD-1 immune checkpoint inhibitor, for patients with advanced, refractory squamous non-small-cell lung cancer (CheckMate 063): a phase 2, single-arm trial. Lancet Oncol.
2015;
16
:
257-65
.
View Article Google Scholar -
Corsello
S.M.,
Barnabei
A.,
Marchetti
P.,
Vecchis
L. De,
Salvatori
R.,
Torino
F.,
Endocrine side effects induced by immune checkpoint inhibitors. J Clin Endocrinol Metab.
2013;
98
(4)
:
1361-75
.
View Article PubMed Google Scholar -
Creelan
B.C.,
Update on immune checkpoint inhibitors in lung cancer. Cancer Control.
2014;
21
:
80-9
.
View Article Google Scholar -
Tokuyasu
T.A.,
Huang
J.D.,
A primer on recent developments in cancer immunotherapy, with a focus on neoantigen vaccines. J Cancer Metastasis Treat.
2018;
4
(1)
:
1-24
.
View Article Google Scholar -
Murray
D.,
Eldershaw
S.A.,
Pearce
H.,
Davies
N.,
McMurray
J.,
Scarisbrick
J.J.,
Moss
P.,
T cell versus T cell; A study of the immune checkpoint landscape in cutaneous T cell lymphoma. Blood.
2017;
130
:
1-6
.
View Article PubMed Google Scholar -
Thommen
D.S.,
Schumacher
T.N.,
T cell dysfunction in cancer. Cancer Cell.
2018;
33
:
547-62
.
View Article Google Scholar -
Grywalska
E.,
Pasiarski
M.,
Góźdź
S.,
Roliński
J.,
Immune-checkpoint inhibitors for combating T-cell dysfunction in cancer. Onco Targets Ther.
2018;
11
:
6505-24
.
View Article Google Scholar -
Ito
A.,
Kondo
S.,
Tada
K.,
Kitano
S.,
Clinical development of immune checkpoint inhibitors. Biomed Res Int.
2015;
2015
:
1-12
.
View Article Google Scholar -
Johnson
D.B.,
Sullivan
R.J.,
Menzies
A.M.,
Immune checkpoint inhibitors in challenging populations. Cancer.
2017;
123
:
1904-11
.
View Article Google Scholar -
Pfannenstiel
L.W.,
Diaz-Montero
C.M.,
Tian
Y.F.,
Scharpf
J.,
Ko
J.S.,
Gastman
B.R.,
Immune-checkpoint blockade opposes CD8+ T-cell suppression in human and murine cancer. Cancer Immunol Res.
2019;
7
(3)
:
510-25
.
View Article Google Scholar -
Pardoll
D.M.,
The blockade of immune checkpoints in cancer immunotherapy. Nat Rev Cancer.
2012;
12
(4)
:
252-64
.
View Article Google Scholar -
Latchman
Y.,
Wood
C.R.,
Chernova
T.,
Chaudhary
D.,
Borde
M.,
Chernova
I.,
PD-L2 is a second ligand for PD-1 and inhibits T cell activation. Nat Immunol.
2001;
2
:
261-8
.
View Article Google Scholar -
Wanchoo
R.,
Karam
S.,
Uppal
N.N.,
Barta
V.S.,
Deray
G.,
Devoe
C.,
Adverse renal effects of immune checkpoint inhibitors: a narrative review. Am J Nephrol.
2017;
45
(2)
:
160-9
.
View Article PubMed Google Scholar -
Agata
Y.,
Kawasaki
A.,
Nishimura
H.,
Ishida
Y.,
Tsubata
T.,
Yagita
H.,
Expression of the PD-1 antigen on the surface of stimulated mouse T and B lymphocytes. Int Immunol.
1996;
8
:
765-72
.
View Article Google Scholar -
Francisco
L.M.,
Sage
P.T.,
Sharpe
A.H.,
The PD-1 pathway in tolerance and autoimmunity. Immunol Rev.
2010;
236
:
219-42
.
View Article Google Scholar -
Ishida
Y.,
Agata
Y.,
Shibahara
K.,
Honjo
T.,
Induced expression of PD-1, a novel member of the immunoglobulin gene superfamily, upon programmed cell death. EMBO J.
1992;
11
(11)
:
3887-95
.
View Article PubMed Google Scholar -
Keir
M.E.,
Butte
M.J.,
Freeman
G.J.,
Sharpe
A.H.,
PD-1 and its ligands in tolerance and immunity. Annu Rev Immunol.
2008;
26
:
677-704
.
View Article Google Scholar -
Okazaki
T.,
Honjo
T.,
PD-1 and PD-1 ligands: from discovery to clinical application. Int Immunol.
2007;
19
:
813-24
.
View Article Google Scholar -
Sury
K.,
Perazella
M.A.,
Shirali
A.C.,
Cardiorenal complications of immune checkpoint inhibitors. Nat Rev Nephrol.
2018;
14
:
571-88
.
View Article Google Scholar -
Walunas
T.L.,
Bakker
C.Y.,
Bluestone
J.A.,
CTLA-4 ligation blocks CD28-dependent T cell activation. J Exp Med.
1996;
183
(6)
:
2541-50
.
View Article PubMed Google Scholar -
Chikuma
S.,
CTLA-4, an essential immune-checkpoint for T-cell activation. Curr Top Microbiol Immuno.
2017;
410
:
99-126
.
View Article Google Scholar -
Leach
D.R.,
Krummel
M.F.,
Allison
J.P.,
Enhancement of antitumor immunity by CTLA-4 blockade. Science.
1996;
271
:
1734-6
.
View Article Google Scholar -
Krummel
M.F.,
Allison
J.P.,
CD28 and CTLA-4 have opposing effects on the response of T cells to stimulation. J Exp Med.
1995;
182
(2)
:
459-65
.
View Article PubMed Google Scholar -
Walunas
T.L.,
Lenschow
D.J.,
Bakker
C.Y.,
Linsley
P.S.,
Freeman
G.J.,
Green
J.M.,
CTLA-4 can function as a negative regulator of T cell activation. Immunity.
1994;
1
(5)
:
405-13
.
View Article PubMed Google Scholar -
Yu
X.,
Huang
X.,
Chen
X.,
Liu
J.,
Wu
C.,
Pu
Q.,
Characterization of a novel anti-human lymphocyte activation gene 3 (LAG-3) antibody for cancer immunotherapy. mAbs.
2019;
11
(6)
:
1139-48
.
View Article PubMed Google Scholar -
Shih
K.,
Arkenau
H.T.,
Infante
J.R.,
Clinical impact of checkpoint inhibitors as novel cancer therapies. Drugs.
2014;
74
:
1993-2014
.
View Article Google Scholar -
Márquez-Rodas
I.,
Cerezuela
P.,
Soria
A.,
Berrocal
A.,
Riso
A.,
González-Cao
M.,
Immune checkpoint inhibitors: therapeutic advances in melanoma. Ann Transl Med.
2015;
3
(18)
:
267
.
View Article Google Scholar -
Nörenberg
J.,
Meggyes
M.,
Jakso
P.,
Miko
E.,
Barakonyi
A.,
TIM-3 and TIM-1 Could Regulate Decidual γδTCR Bright T Cells during Murine Pregnancy. J Immunol Res.
2019;
2019
:
3836942
.
View Article PubMed Google Scholar -
Mintz
M.A.,
Felce
J.H.,
Chou
M.Y.,
Mayya
V.,
Xu
Y.,
Shui
J.W.,
The HVEM-BTLA axis restrains T cell help to germinal center B cells and functions as a cell-extrinsic suppressor in lymphomagenesis. Immunity.
2019;
51
(2)
:
310-23
.
View Article Google Scholar -
Douna
H.,
Amersfoort
J.,
Schaftenaar
F.H.,
Kröner
M.J.,
Kiss
M.B.,
Slütter
B.,
B- and T-lymphocyte attenuator stimulation protects against atherosclerosis by regulating follicular B cells. Cardiovasc Res.
2019;
116
(2)
:
295-305
.
View Article Google Scholar -
Rodriguez-Barbosa
J.I.,
Schneider
P.,
Weigert
A.,
Lee
K.M.,
Kim
T.J.,
Perez-Simon
J.A.,
HVEM, a cosignaling molecular switch, and its interactions with BTLA, CD160 and LIGHT. Cell Mol Immunol.
2019;
16
:
679-82
.
View Article Google Scholar -
Bian
B.,
Fanale
D.,
Dusetti
N.,
Roque
J.,
Pastor
S.,
Chretien
A.S.,
Prognostic significance of circulating PD-1, PD-L1, pan-BTN3As, BTN3A1 and BTLA in patients with pancreatic adenocarcinoma. Oncoimmunology.
2019;
8
(4)
:
e1561120
.
View Article Google Scholar -
Bourque
J.,
Hawiger
D.,
The BTLA–HVEM–CD5 immunoregulatory Axis–an instructive mechanism governing pTreg cell differentiation. Front Immunol.
2019;
10
:
1163
.
View Article Google Scholar -
Grakoui
A.,
Bromley
S.K.,
Sumen
C.,
Davis
M.M.,
Shaw
A.S.,
Allen
P.M.,
The immunological synapse: a molecular machine controlling T cell activation. Science.
1999;
285
(5425)
:
221-7
.
View Article Google Scholar -
Goral
S.,
The three-signal hypothesis of lymphocyte activation targets for immunosuppression. Dial Transplant.
2011;
40
(1)
:
14-6
.
View Article Google Scholar -
Lodygin
D.,
Flügel
A.,
Intravital real-time analysis of T cell activation in health and disease. Cell Calcium.
2017;
64
:
118-29
.
View Article Google Scholar -
Alegre
M.L.,
Frauwirth
K.A.,
Thompson
C.B.,
T-cell regulation by CD28 and CTLA-4. Nat Rev Immuno.
2001;
1
:
220-8
.
View Article Google Scholar -
Curran
M.A.,
Montalvo
W.,
Yagita
H.,
Allison
J.P.,
PD-1 and CTLA-4 combination blockade expands infiltrating T cells and reduces regulatory T and myeloid cells within B16 melanoma tumors. Proc Natl Acad Sci U S A.
2010;
107
:
4175-80
.
View Article Google Scholar -
Hui
E.,
Cheung
J.,
Zhu
J.,
Su
X.,
Taylor
M.J.,
Wallweber
H.A.,
T cell costimulatory receptor CD28 is a primary target for PD-1-mediated inhibition. Science.
2017;
355
:
1428-33
.
View Article Google Scholar -
Ribas
A.,
Wolchok
J.D.,
Cancer immunotherapy using checkpoint blockade. Science.
2018;
359
:
1350-5
.
View Article Google Scholar -
Pilones
K.A.,
Vanpouille-Box
C.,
Demaria
S.,
Combination of radiotherapy and immune checkpoint inhibitors. Semin Radiat Oncol.
2015;
25
:
28-33
.
View Article Google Scholar -
Sharon
E.,
Streicher
H.,
Goncalves
P.,
Chen
H.X.,
Immune checkpoint inhibitors in clinical trials. Chin J Cancer.
2014;
33
(9)
:
434-44
.
View Article PubMed Google Scholar -
Lee
K.M.,
Chuang
E.,
Griffin
M.,
Khattri
R.,
Hong
D.K.,
Zhang
W.,
Molecular basis of T cell inactivation by CTLA-4. Science.
1998;
282
:
2263-6
.
View Article Google Scholar -
Tang
F.,
Zheng
P.,
Tumor cells versus host immune cells: whose PD-L1 contributes to PD-1/PD-L1 blockade mediated cancer immunotherapy?. Cell Biosci.
2018;
8
:
1-8
.
View Article Google Scholar -
Sanmamed
M.F.,
Chen
L.,
A paradigm shift in cancer immunotherapy: from enhancement to normalization. Cell.
2018;
175
:
313-26
.
View Article Google Scholar -
Sharpe
A.H.,
Introduction to checkpoint inhibitors and cancer immunotherapy. Immunol Rev.
2017;
276
:
5-8
.
View Article Google Scholar -
Tumeh
P.C.,
Harview
C.L.,
Yearley
J.H.,
Shintaku
I.P.,
Taylor
E.J.,
Robert
L.,
PD-1 blockade induces responses by inhibiting adaptive immune resistance. Nature.
2014;
515
:
568-71
.
View Article Google Scholar -
Barbee
M.S.,
Ogunniyi
A.,
Horvat
T.Z.,
Dang
T.O.,
Current status and future directions of the immune checkpoint inhibitors ipilimumab, pembrolizumab, and nivolumab in oncology. Ann Pharmacother.
2015;
49
:
907-37
.
View Article Google Scholar -
Constantinidou
A.,
Alifieris
C.,
Trafalis
D.T.,
Targeting Programmed Cell Death -1 (PD-1) and Ligand (PD-L1): A new era in cancer active immunotherapy. Pharmacol Ther.
2019;
194
:
84-106
.
View Article PubMed Google Scholar -
Albring
J.C.,
Inselmann
S.,
Sauer
T.,
Schliemann
C.,
Altvater
B.,
Kailayangiri
S.,
PD-1 checkpoint blockade in patients with relapsed AML after allogeneic stem cell transplantation. Bone Marrow Transplant.
2017;
52
:
317-20
.
View Article Google Scholar -
Taube
J.M.,
Klein
A.,
Brahmer
J.R.,
Xu
H.,
Pan
X.,
Kim
J.H.,
Association of PD-1, PD-1 ligands, and other features of the tumor immune microenvironment with response to anti-PD-1 therapy. Clin Cancer Res.
2014;
20
(19)
:
5064-74
.
View Article PubMed Google Scholar -
Lee
C.K.,
Man
J.,
Lord
S.,
Links
M.,
Gebski
V.,
Mok
T.,
Yang
J.C.,
Checkpoint inhibitors in metastatic EGFR-mutated non-small cell lung cancer-a meta-analysis. J Thorac Oncol.
2017;
12
:
403-407
.
View Article Google Scholar -
Johnson
D.B.,
M.J. Rioth,
L. Horn,
Immune checkpoint inhibitors in NSCLC. Curr Treat Options Oncol.
2014;
15
:
658-69
.
View Article Google Scholar -
Nair
V. Sasidharan,
Elkord
E.,
Immune checkpoint inhibitors in cancer therapy: a focus on T-regulatory cells. Immunol Cell Biol.
2018;
96
:
21-33
.
View Article Google Scholar -
Golden
E.B.,
Demaria
S.,
Schiff
P.B.,
Chachoua
A.,
Formenti
S.C.,
An abscopal response to radiation and ipilimumab in a patient with metastatic non-small cell lung cancer. Cancer Immunol Res.
2013;
1
:
365-72
.
View Article Google Scholar -
Dama
P.,
Tang
M.,
Fulton
N.,
Kline
J.,
Liu
H.,
Gal9/Tim-3 expression level is higher in AML patients who fail chemotherapy. J Immunother Cancer.
2019;
7
(1)
:
1-7
.
View Article PubMed Google Scholar -
Jenkins
R.W.,
Barbie
D.A.,
Flaherty
K.T.,
Mechanisms of resistance to immune checkpoint inhibitors. Br J Cancer.
2018;
118
(1)
:
9-16
.
View Article PubMed Google Scholar -
Brahmer
J.R.,
Pardoll
D.M.,
Immune checkpoint inhibitors: making immunotherapy a reality for the treatment of lung cancer. Cancer Immunol Res.
2013;
1
:
85-91
.
View Article Google Scholar -
Dragomir
M.,
Chen
B.,
Fu
X.,
Calin
G.A.,
Key questions about the checkpoint blockade-are microRNAs an answer?. Cancer Biol Med.
2018;
15
:
103-15
.
View Article Google Scholar -
Delyon
J.,
Brunet-Possenti
F.,
Leonard-Louis
S.,
Arangalage
D.,
Baudet
M.,
Baroudjian
B.,
Immune checkpoint inhibitor rechallenge in patients with immune-related myositis. Ann Rheum Dis.
2018;
78
(11)
:
e129
.
View Article Google Scholar -
Villadolid
J.,
Amin
A.,
Immune checkpoint inhibitors in clinical practice: update on management of immune-related toxicities. Transl Lung Cancer Res.
2015;
4
(5)
:
560-75
.
View Article Google Scholar -
Chen
T.W.,
Razak
A.R.,
Bedard
P.L.,
Siu
L.L.,
Hansen
A.R.,
A systematic review of immune-related adverse event reporting in clinical trials of immune checkpoint inhibitors. Ann Oncol.
2015;
26
(9)
:
1824-9
.
View Article Google Scholar -
Friedman
C.F.,
Proverbs-Singh
T.A.,
Postow
M.A.,
Treatment of the immune-related adverse effects of immune checkpoint inhibitors. JAMA Oncol.
2016;
2
(10)
:
1346-53
.
View Article Google Scholar -
Lyon
A.R.,
Yousaf
N.,
Battisti
N.M.,
Moslehi
J.,
Larkin
J.,
Immune checkpoint inhibitors and cardiovascular toxicity. Lancet Oncol.
2018;
19
(9)
:
e447-58
.
View Article Google Scholar -
Dine
J.,
Gordon
R.,
Shames
Y.,
Kasler
M.K.,
Barton-Burke
M.,
Immune checkpoint inhibitors: an innovation in immunotherapy for the treatment and management of patients with cancer. Asia Pac J Oncol Nurs.
2017;
4
(2)
:
127-35
.
View Article PubMed Google Scholar -
Soularue
E.,
Lepage
P.,
Colombel
J.F.,
Coutzac
C.,
Faleck
D.,
Marthey
L.,
Enterocolitis due to immune checkpoint inhibitors: a systematic review.. Gut.
2018;
67
(11)
:
2056-67
.
View Article Google Scholar -
Torino
F.,
Corsello
S.M.,
Salvatori
R.,
Endocrinological side-effects of immune checkpoint inhibitors. Curr Opin Oncol.
2016;
28
(4)
:
278-87
.
View Article Google Scholar -
Le
D.T.,
Durham
J.N.,
Smith
K.N.,
Wang
H.,
Bartlett
B.R.,
Aulakh
L.K.,
Mismatch repair deficiency predicts response of solid tumors to PD-1 blockade. Science.
2017;
357
(6349)
:
409-13
.
View Article Google Scholar -
González-Rodríguez
E.,
Rodríguez-Abreu
D.,
Immune checkpoint inhibitors: review and management of endocrine adverse events. Oncologist.
2016;
21
:
804-16
.
View Article Google Scholar -
Reddy
H.G.,
Schneider
B.J.,
Tai
A.W.,
Immune Checkpoint Inhibitor-Associated Colitis and Hepatitis. Clin Transl Gastroenterol.
2018;
9
(9)
:
180
.
View Article Google Scholar -
Gibney
G.T.,
Weiner
L.M.,
Atkins
M.B.,
Predictive biomarkers for checkpoint inhibitor-based immunotherapy. Lancet Oncol.
2016;
17
:
e542-51
.
View Article Google Scholar -
Darvin
P.,
Toor
S.M.,
Nair
V. Sasidharan,
Elkord
E.,
Immune checkpoint inhibitors: recent progress and potential biomarkers. Exp Mol Med.
2018;
50
:
1-11
.
View Article Google Scholar -
Vareki
S. Maleki,
Garrigós
C.,
Duran
I.,
Biomarkers of response to PD-1/PD-L1 inhibition. Crit Rev Oncol Hematol.
2017;
116
:
116-24
.
View Article Google Scholar -
Teng
F.,
Meng
X.,
Kong
L.,
Yu
J.,
Progress and challenges of predictive biomarkers of anti PD-1/PD-L1 immunotherapy: A systematic review. Cancer Lett.
2018;
414
:
166-73
.
View Article Google Scholar
Comments
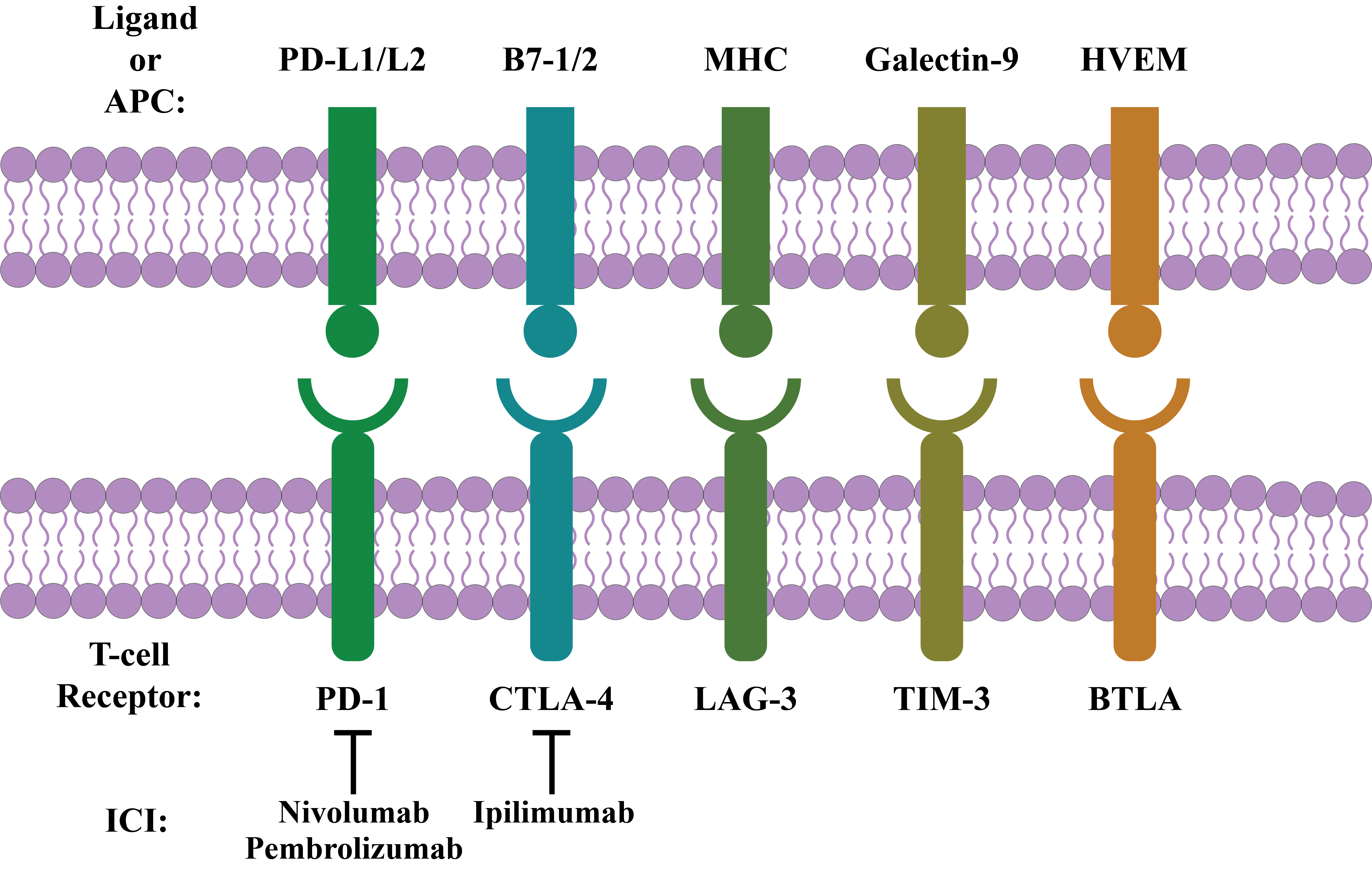
Article Details
Volume & Issue : Vol 9 No 12 (2022)
Page No.: 5455-5464
Published on: 2022-12-31
Citations
Copyrights & License

This work is licensed under a Creative Commons Attribution 4.0 International License.
Search Panel
Pubmed
Google Scholar
Pubmed
Google Scholar
Pubmed
Google Scholar
Pubmed
Search for this article in:
Google Scholar
Researchgate
- HTML viewed - 5979 times
- PDF downloaded - 1457 times
- XML downloaded - 0 times