Abstract
Extracellular vesicles (EVs) are a population of heterogeneous particles that originate from the endosomal system or plasma membrane. Antigen-presenting cells (APCs) produce and release a broad spectrum of EVs involved in the pathogenesis of atherosclerosis. APC-derived EVs contain several bioactive molecules, such as non-coding RNAs, cytokines, chemokines, active proteins, immunomodulatory factors, and growth factors. The review focuses on the role of APC-derived EVs in regulating the transformation of macrophage phenotype, shaping foam cells, driving autophagy and/or inhibiting apoptosis of Th4+ cells, T regulatory cells, endothelial and smooth muscle cells (SMCs), as well as in facilitating oxidative stress in vasculature. APC-derived EVs act as triggers of angiogenesis, neovascularization and inflammation through their participation in microvascular inflammation, angiogenesis, development of atherosclerotic plaques, and modulation of their instability.
Introduction
During the last two decades, extracellular vesicles (EVs) have become a subject of scrutiny in various diseases 1, 2, 3. EVs are defined as heterogeneous groups of membrane-enclosed spherical structures with variable sizes and compositions, including microvesicles, exosomes and apoptotic bodies 4. They are secreted by a wide array of cells, including cardiac myocytes, mature and progenitor endothelial cells, mesenchymal stem cells, immune cells like antigen-presenting cells (APCs), and malignant cells5, 6. Being involved in the transfer of cellular content (e.g. regulatory proteins, hormones, lipids, growth factors, chromatin materials, and microRNAs), EVs play a multifaceted role in cell-to-cell communication, regulation of immune response, tissue reparation, angiogenesis, inflammation and malignancy, via acting as signal transductors which mediate information by internalization of their contents through fusion with the plasma membrane of target cells or through endocytosis7, 8, 9, 10.
Atherosclerosis is defined as a chronic progressive disease of the arterial wall having a long asymptomatic phase. The development of the disease is associated with microvascular inflammation and altered immune reaction due to strong infiltration of intima and sub-intima by oxidized lipids, oxidative stress, proliferative responses from cellular components of intima and media, accumulation of extracellular components with the shaping of atherosclerotic plaques, impaired vasomotion ability, endothelial dysfunction, and thrombosis, ultimately resulting in vascular remodeling and artery obstruction11, 12. Finally, there is a close relationship between a risk of all-cause mortality and incident of fatal and non-fatal cardiovascular (CV) events in the general population and subclinical atherosclerosis13.
Numerous animal studies as well as observational and clinical trials have shown that atherosclerosis arises from immune activation, with several cell-type specific pathways involved. These include macrophage and smooth muscle cell (SMC) phenotypic switching and various inflammatory signaling, such as IL-33/suppression of tumorigenesis 2 (ST2), Ras-Raf-MEK-ERK pathways, and JAK-STAT signaling pathways14, 15, 16, 17. Besides, some chronic infections induced by pathogens such as Helicobacter pylori and herpes zoster can increase the risk of atherosclerosis, mediating the presence of HSP60-specific T lymphocytes in peripheral blood18, 19. However, the innate molecular mechanisms involved in the progression of stable artery lesions to the formation of vulnerable plaques still remain uncertain 20. There is a wide range of evidence regarding the fact that pro-atherogenic factors (e.g. hypoxia, oxidative stress, oxidized lipids, and inflammation) and aggravating factors (e.g. blood flow turbulence, endothelial dysfunction, and vasoconstriction) influence the production of EVs from various cells and promote atherosclerosis21, 22. It has been defined that EVs derived from APCs regulating key steps of disease pathogenesis, such as microvascular inflammation, immunity, cell survival, apoptosis, angiogenesis, thrombosis and autophagy, exert a role in the development of atherosclerotic lesions21, 23. The review is to update the current evidence of the role of EVs derived from immune cells and APCs in the development of atherosclerotic plaque and modulation of their instability.
Definition and nomenclature of extracellular vesicles
The recently updated guideline of the International Society for Extracellular Vesicles (ISEV) on minimal information for studies of extracellular vesicles (MISEV) has defined EVs as "particles naturally released from the cell that is delimited by a lipid bilayer and cannot replicate"24. The nomenclature, main characteristics, and biological function of several subpopulations of EVs are reported in Table 1. Exosomes, microvesicles, and apoptotic bodies are validated to describe several types of particles. Although different subpopulations of EVs have a particular and unique morphological structure, which is a result of their origin, EVs can be substantially distinguished from each other in terms of their components, such as cell organelles (endoplasmic reticulum, Golgi, mitochondrial and nuclear components), cytosolic and cytoskeleton proteins (heat shock proteins, tubulins, moesin, cofilins, actin, myosin, protocadherin, apolipoproteins), lipids (cholesterol, sphingomyelin, ceramide, phosphatidylcholine, ethanolamine, and inositol), eicosanoids, enzymes (phospholipases, matrix metalloproteinases), adhesion molecules (CD44, EPCAM, ICAMs, integrins), genetic materials (chromatin debris, DNA, non-coding and coding RNAs), and growth factors (vascular endothelial growth factor [VEGF], epidermal growth factor [EGF], fibroblast growth factor [FGF] and their receptors [EGF and FGF receptors], interleukins, and other biomolecules)25, 26. Some components (cytosolic and cytoskeleton proteins, growth factors and their receptors, MHC molecules, and adhesive molecules) are constitutively present in various subtypes of EVs. Other components, such as prions and β-amyloid peptides, occur in pathological condition. Moreover, there is no strong correlation between the components, the size, and the number of these particles in circulation27. Despite protein/lipid profiles, as well as nucleic acid species, which could serve as markers for quantitative and compositional characterizations of several subtypes of EVs, there is a need to use other criteria including size, immune phenotype, bilayer morphology, labeling with fluorescent lipids, proteins, or antibodies- to discriminate EVs from each other.
Characteristics | Exosomes | Microvesicles | Apoptotic bodies |
Size (nm) | 40 - 120 | 50 - 1000 | 500 - 5000 |
Mechanisms of formation | Multiple exocytosis from the endosomal system with shaping intraluminal budding of endosomal compartments and intraluminal vesicles prior to the release, which is under control Rab11/35 and Rab27 GTPases, the tetraspanin, ceramide, and the SNARE complex | Blebbing of the plasma membrane due to multiple complex Ca2 + -depending regulatory pathways, which include myosin light chain and depend on Rho-associated kinase I and II, NF-κB, TNF–related apoptosis-inducing ligand, p38 MAPk | Budding from plasma membrane due to caspase-mediated cleavage and activation of Rho-associated kinase I |
Morphology | Cup-shaped | Predominantly heterogeneous | Heterogeneous |
Composition | Proteins, lipids, non-coding and coding RNAs, DNAs, growth factors, MHC molecules, receptors, heparan sulfate proteoglycans including syndecans, complement-binding proteins CD55 and CD59, cystatin C, TNF-α and INF-γ, CD47; heterotrimeric G proteins, transferrin receptor, ADAM10; GPI-anchored 5ʹ nucleotidase CD73, interleukins, FGF-1/2, PDGF | Proteins, lipids, non-coding and coding RNAs, DNAs, growth factors, hormones, and cell organelles, HSC70 (HSPA8), and HSP84, APOA1/2, APOB; APOB100, TGFB1/2; INF-γ, VEGF-A, FGF-1/2, PDGF, EGF, interleukins | Proteins, cell organelles, membrane and cytosolic components, chromatin fragments, histones, non-coding and coding RNAs |
Main biological function | Cell-to-cell communication | Cell-to-cell communication | Facilitate phagocytosis, autophagy, immune response |
Markers | ESCRT components, MFGE8, PDCD6IP, TSG101, flotillin, tetraspanins (CD8, CD63, CD81), SNARE proteins (syntaxin 6 and syntaxin 13). | Integrins, selectins, and CD40 ligand | Annexin V, phosphatidylserine |
EVs derived from immune and antigen-presenting cells: Biological role and function
EVs are produced by immune cells and APCs (macrophages, B cells, dendritic cells) facilitate cell-to-cell communication processes, such as forming immune synapses between APCs and T cells, promoting the delivery of peptide complexes of class II major histocompatibility complex (pMHC-II) molecules, and assisting in the differentiation of T cells into CD4+ or CD8+ T cells. Besides, they can act as antigen-presenting EVs, thereby mediating the initiation, expansion, maintenance, or silencing of adaptive immune responses, while also promoting differentiation of regulatory T lymphocytes, inflammation, and apoptosis 27, 28. Therefore, EVs- especially exosomes, which are secreted by naïve human monocytes/macrophages are involved in regulating the phagocytic activity of activated macrophages as a result of the transfer of interleukin (IL)-10 and transforming growth factor (TGF)-beta. It has been found that numerous microRNAs (-27a, -29b, -125a, -146a, -155, and -222), which are transmitted from naïve human monocytes to macrophages, were powerful triggers for the polarization of macrophages into M2 phenotype 29, 30, 31. Nevertheless, proliferation and differentiation of progenitor endothelial precursors, which are crucial for angiogenesis and neovascularization, are under regulation by APC-derived EVs32. Well-known inductors of cell differentiation, such as circulating oxidase low-density protein and cell-free microRNAs cannot strongly support epigenetic-related regulation of proliferative activity of resident cells in the vasculature. Several microRNAs (such as microRNA-128, microRNA-128-1, microRNA-148a, microRNA-130b and microRNA-301b) are cargoes for EVs and act as specifically-designed core post-transcriptional regulators of target genes involved in cellular lipid homeostasis, microvascular inflammation, and energy metabolism. These target genes include LDL receptor, ATP-binding cassette transporter A1, sirtuin 1, and insulin receptor substrate 133, 34, 35. Thus, specific proteomic, transcriptomic, and lipidomic profiles of EVs secreted by immune cells and APCs are engaged in control of migration, proliferation and differentiation of recipient cells.
Immune and APC-derived EVs in atherosclerosis
Previous preclinical and clinical studies have revealed that EVs are associated with the presence, progressiveness, and severity of atherosclerosis36, 37, 38. Indeed, circulating levels of EVs have been found to be increased in atherosclerosis, and EVs were EVs involved in key stages of atherosclerosis progression, such as accumulation of lipid, thickness of intima, proliferative response from SMCs, promotion of vascular media and calcification, plaque shaping and progression, and thrombus formation after plaque rupture36. The proliferation and migration of macrophages, epithelial cells and vascular SMCs, as well as the transformation of macrophages into foam cells, are all essential elements for the formation of atherosclerotic plaques and acceleration of atherosclerosis37. Activated macrophages can interact with vascular SMCs through exosomes and stimulate them to migrate and adhere to the intima. EVs derived from foam cells have been demonstrated to stimulate vascular SMC migration and activate extracellular signal-regulated kinase (ERK) pathways, enabling the progression of aggravated lesions38. Oxidized low-density lipoproteins, from internalization into EVs, can be transferred from foam cells to vascular SMCs and endothelial cells. The resident precursors act as destructive stimuli, inducing oxidative stress and disintegration of the endothelial barrier. It has been established that several transcriptional factors, such as Krüppel-like factor-5 (KLF5), JunD (a member of the activated protein-1 family of transcription factors), and nuclear factor erythroid 2-related factor 2 (Nrf2), were found to be incorporated into EVs derived from macrophages. Consequently, EVs mediating the proliferation and migration of vascular SMCs via supply of transcription factors- play a pivotal role in adverse vascular remodeling and atherosclerotic plaque shaping while suppressing oxidative homeostasis in target cells of the vasculature; however, they can attenuate angiopoietin capacity38, 39.
There is convincing evidence that a large number of non-coding RNAs, including microRNA-146a, microRNA-128, microRNA-185, microRNA-199a-5p, microRNA-365 and microRNA-503, are transmitted between immune cells and target somatic cells (such as epithelial cells, progenitor endothelial cells and SMCs), promoting specific signals for suppression of aerobic glycolysis, promoting macrophage polarization, and decreasing cell migration39, 40. Interestingly, delivery of microRNA-146a from macrophage-released EVs repressed the expression of target genes of insulin-like growth factor 2 mRNA-binding protein 1 (IGF2BP1) and human antigen R or ELAV-like RNA-binding protein 1 (HuR) in naïve macrophages40. These genes activate downstream cascades, including that of NLRP3 inflammasome, and support Toll-like receptor (TLR) signaling and endoplasmic reticulum stress responses, thereby hampering recruitment of circulating monocytes and macrophages into the vascular intima41. Nonetheless, microRNA-223 contained in macrophage-released EVs was found to be a powerful trigger for macrophage turn-over into foam cells in atherosclerotic plaques 41. Moreover, microRNA-199a-5p can exert its effect by targeting Klotho, which induces polarization of M2 macrophages through the TLR-4 pathway42.
There is a large body of evidence that shows macrophages can secrete so-called atherogenic exosomes containing microRNAs (-21-3p, -133a, -141-3p) to mediate cell-to-cell crosstalk and encourage pro-atherogenic phenotypes of vascular SMCs43, 44, 45. Indeed, EVs enriched by microRNA-21-3p and derived from plaque-resident macrophages increase vascular SMC migration and proliferation via their phosphatase and tension homology43. The package of microRNA-133a in macrophage secretome is associated with a negative regulation of cell proliferation, inflammatory factor secretion, and apoptosis in vascular wall and plaque by modulating FGF-144. Animal studies have revealed that microRNA-141-3p deletion reverses the positive effects on vascular SMCs via long non-coding RNA-taurine-upregulated gene 145. Thus, macrophages modulate pro-inflammatory and pro-atherogenic phenotypes in recipient cells via the secretion of EVs containing microRNAs.
Additionally, EVs are a powerful messenger for signals from infected cells to naïve cells. EVs released from virus-infected cells deliver viral RNA to dendritic cells and macrophages, thereby activating pattern recognition receptors (PRRs) on recipient cells, resulting in the expression of type I interferons and pro-inflammatory cytokines46, 47. On the other hand, exosome-mediated secretion of a multitude of immunoregulatory proteins from APCs has been demonstrated; moreover, EVs can promote inflammasome creation and release as an alternative to caspase-148, 49. Consequently, EVs can indirectly modulate the non-canonical secretion of pro-inflammatory cytokines IL-1β and IL-18 as a package of the inflammasome. Moreover, TLR-9 activated macrophages can secrete EVs that ensure transport of various nucleic acids and CpG oligodeoxynucleotides to naïve macrophages and induce them to release chemokines and TNF-α50, 51. Besides, one of the largest stress-induced proteins and molecular chaperones- glucose-regulated protein 170 (Grp170)- is highly responsible for the internalization of CpG oligodeoxynucleotide package and facilitates synergistic activation through GTP-binding protein Ras and MyD88-dependent signaling (MyD88/IRAK/TRAF6 kinases cascade, ERK/JNK/NF-kappaB), which ensures a subsequent enhancement in production of pro-inflammatory cytokines and nitric oxide 52, 53, 54. These molecules were previously defined as triggers for proliferative responses from vascular SMCs, epithelial and endothelial cells, and resident macrophages55.
Apoptotic APC-derived EVs have been found to be core players in contributing to macrophage-mediated production of TGF-beta in vitro and in vivo56. In extracellular immune surveillance, APC-derived EVs also interacted with secreted phospholipases to generate eicosanoids, regulating the transfer of cargo into a cellular recipient57. Eicosanoids are involved in various biological functions, including modulation or modification of phenotype of the recipient cells, such as SMCs, macrophages, and endothelial progenitor cells; distal immune responses and proliferative responses from SMCs can also be modulated 58, 59.
Since EVs contain a wide spectrum of lipids, the final metabolic effect on target cells depends on lipids that enrich EVs and the immune phenotype of EVs. For instance, di-saturated phospholipids that are embarked by exosomes enhance their membrane rigidity and facilitate binding with circulating IgM-type immunoglobulins60. There are additional specific eliminating proteins that may favor the clearance of circulating immune complexes, IgM antibodies, and apoptotic cells by exosomes. Indeed, the phospholipase iPLA2, which is specifically associated with the endosomal and exosomal membranes, can be activated by reactive oxygen species and mediates lysophosphatidylcholine synthesis. It is recognized by IgM antibodies on the surface of EVs and specifically binds with apoptotic cells, leading to their removal from circulation 61, 62. Because apoptotic cells derived from macrophages and other APCs can suppress pro-inflammatory and pro-immunogenic reactions through their cargo contents, altered elimination of these EVs from peripheral blood with exosomes is considered as an impaired endogenous tissue-protective mechanism63, 64. In fact, apoptotic cells can induce 15-lipoxygenase and 15-hydroxyeicosatetraenoic acid production, which potentiate the anti-inflammatory pathway through peroxisome proliferator-activated receptor-gamma and lipoxin A4 production, leading to maintenance of vascular integrity and prevention against atherosclerosis65.
Another pathophysiological mechanism by which exosomes released from oxidized low-density lipoprotein-stimulated macrophages influence atherosclerosis development and progression is via induction of neutrophil extracellular traps (NETs)66. Overall, NETosis is a unique cell death mechanism that is a crucial component of the adaptive immune response, linking microvascular inflammation with atherosclerosis67, 68. Exosomal microRNA-146a secreted by activated macrophages promote the generation of intracellular reactive oxygen species and NET release via targeting superoxide dismutase 2 66. Therefore, activation of endothelial cells with APC-derived EVs can promote them to secrete exosomes embarked with microRNA-505, oxidized low-density lipoprotein, and metastasis-associated lung adenocarcinoma transcript 1 (MALAT1). Furthermore, MALAT1 and microRNA-505 containing various endothelial cell-derived EVs (mainly exosomes) are able to initiate the formation of NETs and plaque resident dendritic cell maturation, which in turn deteriorate atherosclerosis68, 69, 70. Indeed, transcripts of long non-coding RNAs found in EVs related to atherosclerosis have included many molecules, such as ANRIL, SENCR, CoroMarker, LIPCAR, HIF1α-AS1, LncRNA H19, APPAT, KCNQ1OT1, LncPPARδ, LincRNA-p21, MALAT1, MIAT, and UCA171. Some of them, such as CoroMarker, have predictive value for coronary artery disease72, 73. On the other hand, animal studies have revealed that exosomal MALAT1 enhances autophagy and survival in oxidized low-density lipoprotein-treated human umbilical vein endothelial cells through suppression of microRNA-216a-5p, and that regulation of Beclin-1 expression can lead to vascular protection74. Consequently, the secretome of APC-derived EVs can play a dual role in atherosclerosis development and progression, depending on the compounds incorporated in the EVs75.
Future investigations
Since circulating EVs are enriched with various subtypes of biologically active molecules and can be derived from individual APCs, single EV analysis might have practical utility to identify patients at high-risk for atherosclerosis by evaluating EV numbers and the cargo composition. Perhaps, extensive clinical studies are required to evaluate whether EVs derived from cells, including APCs, could serve as potential biomarkers of subclinical atherosclerosis. Finally, an exosome-based therapeutic strategy can also be used to attenuate atherosclerotic heart disease and promote cardiovascular regeneration.
Conclusion
EVs derived from APCs play a central role in accelerating atherosclerosis through their participation in microvascular inflammation, angiogenesis, coagulation, and NETosis. While limited, there is strong evidence in the literature for APC-derived EVs as potential diagnostic and predictive markers but this requires further investigations. Large clinical trials can help deepen our understanding of APC-derived EVs as potential surrogate biomarkers of atherosclerosis-associated diseases.
Abbreviations
Apo: apo-lipoproteins
CD: cluster of differentiation
CV: cardiovascular
CVD: cardiovascular disease
EGF: Epidermal Growth Factor
ESCRT: endosomal sorting complex required for transport
FGF: fibroblast growth factor
HSP: heat shock proteins
INF: interferon
MAPk: mitogen-activated protein kinase
MFGE8: milk fat globule-EGF factor 8 protein
NF-κB: nuclear factor-κB
PDCD6IP: programmed cell death 6 interacting protein
PDGF: platelet-derived growth factor
SNARE: soluble N-ethylmaleimide-sensitive attachment protein receptor
TNF: tumor necrosis factor
TSG101: tumor susceptibility gene 101 protein
VEGF-A: vascular endothelial growth factor-A
Acknowledgments
None.
Author’s contributions
Berezin AE and Berezin AA have equal responsible for the paper. All authors read and approved the final manuscript.
Funding
None.
Availability of data and materials
Not applicable.
Ethics approval and consent to participate
Not applicable.
Consent for publication
Not applicable.
Competing interests
The authors declare that they have no competing interests.
References
-
Turturici G, Tinnirello R, Sconzo G, Geraci F. Extracellular membrane vesicles as a mechanism of cell-to-cell communication: advantages and disadvantages. Am J Physiol Cell Physiol. 2014; 306(7):C621-33.
.
View Article PubMed Google Scholar -
Greening DW, Simpson RJ. Understanding extracellular vesicle diversity - current status. Expert Rev Proteomics. 2018; 15(11):887-910.
.
View Article PubMed Google Scholar -
Berezin AE. Microparticles in Chronic Heart Failure. Adv Clin Chem. 2017; 81:1-41.
.
View Article PubMed Google Scholar -
Abels ER, Breakefield XO. Introduction to Extracellular Vesicles: Biogenesis, RNA Cargo Selection, Content, Release, and Uptake. Cell Mol Neurobiol. 2016; 36(3):301-12.
.
View Article PubMed Google Scholar -
Zhang B, Yeo RW, Tan KH, Lim SK. Focus on Extracellular Vesicles: Therapeutic Potential of Stem Cell-Derived Extracellular Vesicles. Int J Mol Sci. 2016; 17(2):174.
.
View Article PubMed Google Scholar -
Ciardiello C, Cavallini L, Spinelli C, Yang J, Reis-Sobreiro M, de Candia P, Minciacchi VR, Di Vizio D. Focus on Extracellular Vesicles: New Frontiers of Cell-to-Cell Communication in Cancer. Int J Mol Sci. 2016; 17(2):175.
.
View Article PubMed Google Scholar -
Mathieu M, Martin-Jaular L, Lavieu G, Théry C. Specificities of secretion and uptake of exosomes and other extracellular vesicles for cell-to-cell communication. Nat Cell Biol. 2019; 21(1):9-17.
.
View Article PubMed Google Scholar -
D'Souza-Schorey C, Schorey JS. Regulation and mechanisms of extracellular vesicle biogenesis and secretion. Essays Biochem. 2018;62(2):125-133.
.
View Article PubMed Google Scholar -
Todorova D, Simoncini S, Lacroix R, Sabatier F, Dignat-George F. Extracellular Vesicles in Angiogenesis. Circ Res. 2017; 120(10):1658-1673.
.
View Article PubMed Google Scholar -
Hafiane A, Daskalopoulou SS. Extracellular vesicles characteristics and emerging roles in atherosclerotic cardiovascular disease. Metabolism. 2018; 85:213-222.
.
View Article PubMed Google Scholar -
Libby P, Ridker PM, Hansson GK. Progress and challenges in translating the biology of atherosclerosis. Nature. 2011; 473(7347):317-25.
.
View Article PubMed Google Scholar -
Tada H, Nohara A, Inazu A, Mabuchi H, Kawashiri MA. Remnant lipoproteins and atherosclerotic cardiovascular disease. Clin Chim Acta. 2019;490:1-5.
.
View Article PubMed Google Scholar -
Cai X, Zhang Y, Li M, Wu JH, Mai L, Li J, Yang Y, Hu Y, Huang Y. Association between prediabetes and risk of all cause mortality and cardiovascular disease: updated meta-analysis. BMJ. 2020; 370:m2297.
.
View Article PubMed Google Scholar -
Bäck M, Hansson GK. Anti-inflammatory therapies for atherosclerosis. Nat Rev Cardiol. 2015; 12(4):199-211.
.
View Article PubMed Google Scholar -
Winkels H, Ehinger E, Vassallo M, Buscher K, Dinh HQ, Kobiyama K, Hamers AAJ, Cochain C, Vafadarnejad E, Saliba AE, Zernecke A, Pramod AB, Ghosh AK, Anto Michel N, Hoppe N, Hilgendorf I, Zirlik A, Hedrick CC, Ley K, Wolf D. Atlas of the Immune Cell Repertoire in Mouse Atherosclerosis Defined by Single-Cell RNA-Sequencing and Mass Cytometry. Circ Res. 2018; 122(12):1675-1688.
.
View Article Google Scholar -
Gomez D, Owens GK. Smooth muscle cell phenotypic switching in atherosclerosis. Cardiovasc Res. 2012; 95(2):156-64.
.
View Article PubMed Google Scholar -
Aimo A, Migliorini P, Vergaro G, Franzini M, Passino C, Maisel A, Emdin M. The IL-33/ST2 pathway, inflammation and atherosclerosis: Trigger and target? Int J Cardiol. 2018; 267:188-192.
.
View Article PubMed Google Scholar -
Ayada K, Yokota K, Kobayashi K, Shoenfeld Y, Matsuura E, Oguma K. Chronic infections and atherosclerosis. Ann N Y Acad Sci. 2007; 1108:594-602.
.
View Article PubMed Google Scholar -
Chmiela M, Gajewski A, Rudnicka K. Helicobacter pylori vs coronary heart disease - searching for connections. World J Cardiol. 2015;7(4):187-203.
.
View Article PubMed Google Scholar -
Millon A, Canet-Soulas E, Boussel L, Fayad Z, Douek P. Animal models of atherosclerosis and magnetic resonance imaging for monitoring plaque progression. Vascular. 2014; 22(3):221-37.
.
View Article PubMed Google Scholar -
Deng W, Tang T, Hou Y, Zeng Q, Wang Y, Fan W, Qu S. Extracellular vesicles in atherosclerosis. Clin Chim Acta. 2019; 495:109-117.
.
View Article PubMed Google Scholar -
Peng M, Liu X, Xu G. Extracellular Vesicles as Messengers in Atherosclerosis. J Cardiovasc Transl Res. 2020; 13(2):121-130. doi: 10.1007/s12265-019-09923-z. .
.
View Article PubMed Google Scholar -
Ramis
J.M.,
Extracellular Vesicles in Cell Biology and Medicine. Sci Rep. 2020; 10, 8667.
.
View Article PubMed Google Scholar -
C. Théry,
Minimal information for studies of extracellular vesicles 2018 (MISEV2018): a position statement of the International Society for Extracellular Vesicles and update of the MISEV2014 guidelines. J Extracell Vesicles. 2018;
7
(1)
:
1535750
.
View Article PubMed Google Scholar -
Boilard E. Extracellular vesicles and their content in bioactive lipid mediators: more than a sack of microRNA. J Lipid Res. 2018; 59(11):2037-2046.
.
View Article PubMed Google Scholar -
Turchinovich A, Drapkina O, Tonevitsky A. Transcriptome of Extracellular Vesicles: State-of-the-Art. Front Immunol. 2019.
.
View Article PubMed Google Scholar -
Witwer KW, Théry C. Extracellular vesicles or exosomes? On primacy, precision, and popularity influencing a choice of nomenclature. J Extracell Vesicles. 2019; 8(1):1648167.
.
View Article PubMed Google Scholar -
Lindenbergh MFS, Stoorvogel W. Antigen Presentation by Extracellular Vesicles from Professional Antigen-Presenting Cells. Annu Rev Immunol. 2018; 36:435-459.
.
View Article PubMed Google Scholar -
Saha B, Momen-Heravi F, Kodys K, Szabo G. MicroRNA Cargo of Extracellular Vesicles from Alcohol-exposed Monocytes Signals Naive Monocytes to Differentiate into M2 Macrophages. J Biol Chem. 2016; 291(1):149-59.
.
View Article PubMed Google Scholar -
Graff JW, Dickson AM, Clay G, McCaffrey AP, Wilson ME. Identifying functional microRNAs in macrophages with polarized phenotypes. J Biol Chem. 2012; 287(26):21816-25.
.
View Article PubMed Google Scholar -
Mosser DM, Edwards JP. Exploring the full spectrum of macrophage activation. Nat Rev Immunol. 2008; 8(12):958-69.
.
View Article PubMed Google Scholar -
György B, Szabó TG, Pásztói M, Pál Z, Misják P, Aradi B, László V, Pállinger E, Pap E, Kittel A, Nagy G, Falus A, Buzás EI. Membrane vesicles, current state-of-the-art: emerging role of extracellular vesicles. Cell Mol Life Sci. 2011; 68(16):2667-88.
.
View Article PubMed Google Scholar -
Choi DS, Kim DK, Kim YK, Gho YS. Proteomics, transcriptomics and lipidomics of exosomes and ectosomes. Proteomics. 2013;13(10-11):1554-71.
.
View Article PubMed Google Scholar -
Wagschal A, Najafi-Shoushtari SH, Wang L, Goedeke L, Sinha S, deLemos AS, Black JC, Ramírez CM, Li Y, Tewhey R, Hatoum I, Shah N, Lu Y, Kristo F, Psychogios N, Vrbanac V, Lu YC, Hla T, de Cabo R, Tsang JS, Schadt E, Sabeti PC, Kathiresan S, Cohen DE, Whetstine J, Chung RT, Fernández-Hernando C, Kaplan LM, Bernards A, Gerszten RE, Näär AM. Genome-wide identification of microRNAs regulating cholesterol and triglyceride homeostasis. Nat Med. 2015; 21(11):1290-7.
.
View Article PubMed Google Scholar -
Chen F, Chen J, Yang L, Liu J, Zhang X, Zhang Y, Tu Q, Yin D, Lin D, Wong PP, Huang D, Xing Y, Zhao J, Li M, Liu Q, Su F, Su S, Song E. Extracellular vesicle-packaged HIF-1α-stabilizing lncRNA from tumour-associated macrophages regulates aerobic glycolysis of breast cancer cells. Nat Cell Biol. 2019; 21(4):498-510.
.
View Article PubMed Google Scholar -
Deng W, Tang T, Hou Y, Zeng Q, Wang Y, Fan W, Qu S. Extracellular vesicles in atherosclerosis. Clin Chim Acta. 2019; 495:109-117.
.
View Article PubMed Google Scholar -
Peng M, Liu X, Xu G. Extracellular Vesicles as Messengers in Atherosclerosis. J Cardiovasc Transl Res. 2020;13(2):121-130. .
.
View Article PubMed Google Scholar -
Berezin A. Neutrophil extracellular traps: The core player in vascular complications of diabetes mellitus. Diabetes Metab Syndr. 2019;13(5):3017-3023.
.
View Article PubMed Google Scholar -
Nguyen MA, Karunakaran D, Geoffrion M, Cheng HS, Tandoc K, Perisic Matic L, Hedin U, Maegdefessel L, Fish JE, Rayner KJ. Extracellular Vesicles Secreted by Atherogenic Macrophages Transfer MicroRNA to Inhibit Cell Migration. Arterioscler Thromb Vasc Biol. 2018; 38(1):49-63.
.
View Article PubMed Google Scholar -
Duewell P, Kono H, Rayner KJ, Sirois CM, Vladimer G, Bauernfeind FG, Abela GS, Franchi L, Nuñez G, Schnurr M, Espevik T, Lien E, Fitzgerald KA, Rock KL, Moore KJ, Wright SD, Hornung V, Latz E. NLRP3 inflammasomes are required for atherogenesis and activated by cholesterol crystals. Nature. 2010; 464(7293):1357-61. doi: 10.1038/nature08938. Erratum in: Nature. 2010; 466(7306): 652.
.
View Article PubMed Google Scholar -
Ismail N, Wang Y, Dakhlallah D, Moldovan L, Agarwal K, Batte K, Shah P, Wisler J, Eubank TD, Tridandapani S, Paulaitis ME, Piper MG, Marsh CB. Macrophage microvesicles induce macrophage differentiation and miR-223 transfer. Blood. 2013; 121(6):984-95.
.
View Article PubMed Google Scholar -
Jia Y, Zheng Z, Xue M, Zhang S, Hu F, Li Y, Yang Y, Zou M, Li S, Wang L, Guan M, Xue Y. Extracellular Vesicles from Albumin-Induced Tubular Epithelial Cells Promote the M1 Macrophage Phenotype by Targeting Klotho. Mol Ther. 2019; 27(8):1452-1466.
.
View Article PubMed Google Scholar -
Zhu J, Liu B, Wang Z, Wang D, Ni H, Zhang L, Wang Y. Exosomes from nicotine-stimulated macrophages accelerate atherosclerosis through miR-21-3p/PTEN-mediated VSMC migration and proliferation. Theranostics. 2019; 9(23):6901-6919.
.
View Article PubMed Google Scholar -
Zhang L, Cheng H, Yue Y, Li S, Zhang D, He R. TUG1 knockdown ameliorates atherosclerosis via up-regulating the expression of miR-133a target gene FGF1. Cardiovasc Pathol. 2018; 33:6-15.
.
View Article PubMed Google Scholar -
Tang Y, Hu J, Zhong Z, Liu Y, Wang Y. Long Noncoding RNA TUG1 Promotes the Function in ox-LDL-Treated HA-VSMCs via miR-141-3p/ROR2 Axis. Cardiovasc Ther. 2020 May 29; 2020: 6758934.
.
View Article PubMed Google Scholar -
Okamoto M, Tsukamoto H, Kouwaki T, Seya T, Oshiumi H. Recognition of Viral RNA by Pattern Recognition Receptors in the Induction of Innate Immunity and Excessive Inflammation During Respiratory Viral Infections. Viral Immunol. 2017; 30(6): 408-420.
.
View Article PubMed Google Scholar -
Kouwaki T, Okamoto M, Tsukamoto H, Fukushima Y, Oshiumi H. Extracellular Vesicles Deliver Host and Virus RNA and Regulate Innate Immune Response. Int J Mol Sci. 2017;18(3):666.
.
View Article PubMed Google Scholar -
Cypryk W, Nyman TA, Matikainen S. From Inflammasome to Exosome-Does Extracellular Vesicle Secretion Constitute an Inflammasome-Dependent Immune Response? Front Immunol. 2018;9:2188.
.
View Article PubMed Google Scholar -
Tabas I, Garcia-Cardena G, Owens GK. Recent insights into the cellular biology of atherosclerosis. The Journal of Cell Biology. 2015; 209(1):13-22.
.
View Article PubMed Google Scholar -
Zhang Y, Jin X, Liang J, Guo Y, Sun G, Zeng X, Yin H. Extracellular vesicles derived from ODN-stimulated macrophages transfer and activate Cdc42 in recipient cells and thereby increase cellular permissiveness to EV uptake. Sci Adv. 2019; 5(7): eaav1564.
.
View Article PubMed Google Scholar -
Calderwood SK, Gong J, Murshid A. Extracellular HSPs: The Complicated Roles of Extracellular HSPs in Immunity. Front Immunol. 2016;7:159.
.
View Article Google Scholar -
Zuo D, Yu X, Guo C, Yi H, Chen X, Conrad DH, Guo TL, Chen Z, Fisher PB, Subjeck JR, Wang XY. Molecular chaperoning by glucose-regulated protein 170 in the extracellular milieu promotes macrophage-mediated pathogen sensing and innate immunity. FASEB J. 2012; 26(4):1493-505. doi: 10.1096/fj.11-197707.
.
View Article PubMed Google Scholar -
Xu H, An H, Yu Y, Zhang M, Qi R, Cao X. Ras participates in CpG oligodeoxynucleotide signaling through association with toll-like receptor 9 and promotion of interleukin-1 receptor-associated kinase/tumor necrosis factor receptor-associated factor 6 complex formation in macrophages. J Biol Chem. 2003; 278(38):36334-40.
.
View Article PubMed Google Scholar -
Yeo SJ, Yoon JG, Yi AK. Myeloid differentiation factor 88-dependent post-transcriptional regulation of cyclooxygenase-2 expression by CpG DNA: tumor necrosis factor-alpha receptor-associated factor 6, a diverging point in the Toll-like receptor 9-signaling. J Biol Chem. 2003; 278(42):40590-600.
.
View Article PubMed Google Scholar -
Hafiane A, Daskalopoulou SS. Extracellular vesicles characteristics and emerging roles in atherosclerotic cardiovascular disease. Metabolism. 2018; 85:213-222.
.
View Article PubMed Google Scholar -
Chen H, Kasagi S, Chia C, Zhang D, Tu E, Wu R, Zanvit P, Goldberg N, Jin W, Chen W. Extracellular Vesicles from Apoptotic Cells Promote TGFβ Production in Macrophages and Suppress Experimental Colitis. Sci Rep. 2019; 9(1): 5875.
.
View Article PubMed Google Scholar -
Taha EA, Ono K, Eguchi T. Roles of Extracellular HSPs as Biomarkers in Immune Surveillance and Immune Evasion. Int J Mol Sci. 2019;20(18):4588.
.
View Article PubMed Google Scholar -
Boilard E. Extracellular vesicles and their content in bioactive lipid mediators: more than a sack of microRNA. J Lipid Res. 2018; 59(11):2037-2046.
.
View Article PubMed Google Scholar -
Record M, Silvente-Poirot S, Poirot M, Wakelam MJO. Extracellular vesicles: lipids as key components of their biogenesis and functions. J Lipid Res. 2018; 59(8):1316-1324.
.
View Article PubMed Google Scholar -
Blanc L, Barres C, Bette-Bobillo P, Vidal M. Reticulocyte-secreted exosomes bind natural IgM antibodies: involvement of a ROS-activatable endosomal phospholipase iPLA2. Blood. 2007 Nov 1; 110(9):3407-16.
.
View Article PubMed Google Scholar -
Laulagnier K, Motta C, Hamdi S, Roy S, Fauvelle F, Pageaux JF, Kobayashi T, Salles JP, Perret B, Bonnerot C, Record M. Mast cell- and dendritic cell-derived exosomes display a specific lipid composition and an unusual membrane organization. Biochem J. 2004;380(Pt 1):161-171.
.
View Article PubMed Google Scholar -
Blanc L, Vidal M. Reticulocyte membrane remodeling: contribution of the exosome pathway. Curr Opin Hematol. 2010; 17(3):177-83.
.
View Article PubMed Google Scholar -
Freire-de-Lima CG, Xiao YQ, Gardai SJ, Bratton DL, Schiemann WP, Henson PM. Apoptotic cells, through transforming growth factor-beta, coordinately induce anti-inflammatory and suppress pro-inflammatory eicosanoid and NO synthesis in murine macrophages. J Biol Chem. 2006 Dec 15; 281(50): 38376-84.
.
View Article PubMed Google Scholar -
Wang Y, Subramanian M, Yurdagul A Jr, Barbosa-Lorenzi VC, Cai B, de Juan-Sanz J, Ryan TA, Nomura M, Maxfield FR, Tabas I. Mitochondrial Fission Promotes the Continued Clearance of Apoptotic Cells by Macrophages. Cell. 2017; 171(2):331-345.e22.
.
View Article PubMed Google Scholar -
Koelwyn GJ, Corr EM, Erbay E, Moore KJ. Regulation of macrophage immunometabolism in atherosclerosis. Nat Immunol. 2018; 19(6):526-537.
.
View Article PubMed Google Scholar -
Zhang YG, Song Y, Guo XL, Miao RY, Fu YQ, Miao CF, Zhang C. Exosomes derived from oxLDL-stimulated macrophages induce neutrophil extracellular traps to drive atherosclerosis. Cell Cycle. 2019; 18(20):2674-2684.
.
View Article PubMed Google Scholar -
Berezin A. Neutrophil extracellular traps: The core player in vascular complications of diabetes mellitus. Diabetes Metab Syndr. 2019; 13(5):3017-3023.
.
View Article PubMed Google Scholar -
Gao H, Wang X, Lin C, An Z, Yu J, Cao H, Fan Y, Liang X. Exosomal MALAT1 derived from ox-LDL-treated endothelial cells induce neutrophil extracellular traps to aggravate atherosclerosis. Biol Chem. 2020; 401(3):367-376.
.
View Article PubMed Google Scholar -
Chen L, Hu L, Li Q, Ma J, Li H. Exosome-encapsulated miR-505 from ox-LDL-treated vascular endothelial cells aggravates atherosclerosis by inducing NET formation. Acta Biochim Biophys Sin (Shanghai). 2019; 51(12):1233-1241.
.
View Article PubMed Google Scholar -
Li H, Zhu X, Hu L, Li Q, Ma J, Yan J. Loss of exosomal MALAT1 from ox-LDL-treated vascular endothelial cells induces maturation of dendritic cells in atherosclerosis development. Cell Cycle. 2019; 18(18):2255-2267.
.
View Article PubMed Google Scholar -
Lu S, Liang Q, Huang Y, Meng F, Liu J. Definition and review on a category of long non-coding RNA: Atherosclerosis-associated circulating lncRNA (ASCLncRNA). PeerJ. 2020; 8:e10001.
.
View Article PubMed Google Scholar -
Yang Y, Cai Y, Wu G, Chen X, Liu Y, Wang X, Yu J, Li C, Chen X, Jose PA, Zhou L, Zeng C. Plasma long non-coding RNA, CoroMarker, a novel biomarker for diagnosis of coronary artery disease. Clin Sci (Lond). 2015;129(8):675-85.
.
View Article PubMed Google Scholar -
Zhong W, Deng Q, Deng X, Zhong Z, Hou J. Long non-coding RNA expression profiles in peripheral blood mononuclear cells of patients with coronary artery disease. J Thorac Dis. 2020; 12(11):6813-6825.
.
View Article PubMed Google Scholar -
Wang K, Yang C, Shi J, Gao T. Ox-LDL-induced lncRNA MALAT1 promotes autophagy in human umbilical vein endothelial cells by sponging miR-216a-5p and regulating Beclin-1 expression. Eur J Pharmacol. 2019; 858: 172338.
.
View Article PubMed Google Scholar -
Wang Y, Xie Y, Zhang A, Wang M, Fang Z, Zhang J. Exosomes: An emerging factor in atherosclerosis. Biomed Pharmacother. 2019; 115:108951.
.
View Article PubMed Google Scholar
Comments
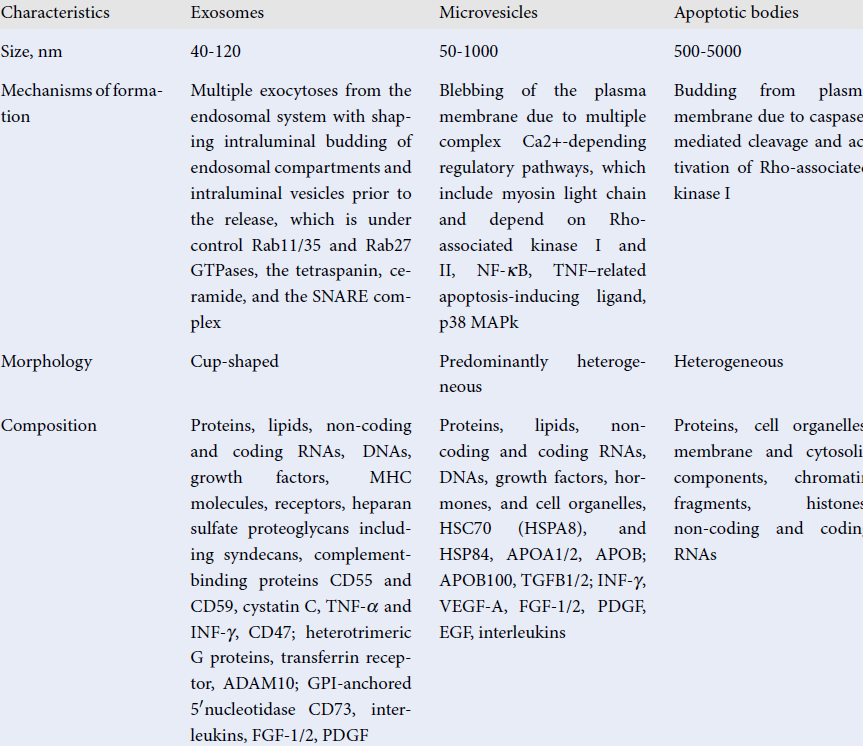
Downloads
Article Details
Volume & Issue : Vol 8 No 3 (2021)
Page No.: 4258-4266
Published on: 2021-03-31
Citations
Copyrights & License

This work is licensed under a Creative Commons Attribution 4.0 International License.
Search Panel
- HTML viewed - 7262 times
- Download PDF downloaded - 1828 times
- View Article downloaded - 0 times